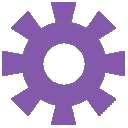
Browsing Pathways
Showing 366911 -
366920 of 605359 pathways
PathBank ID | Pathway Name and Description | Pathway Class | Chemical Compounds | Proteins |
---|---|---|---|---|
SMP0438432![]() |
Fructose MetabolismBacteroides thetaiotaomicron VPI-5482
Fructose metabolism begins with the transport of Beta-D-fructofuranose through a fructose PTS permease, resulting in a Beta-D-fructofuranose 1-phosphate. This compound is phosphorylated by an ATP driven 1-phosphofructokinase resulting in a fructose 1,6-biphosphate. This compound can either react with a fructose bisphosphate aldolase class 1 resulting in D-glyceraldehyde 3-phosphate and a dihydroxyacetone phosphate or through a fructose biphosphate aldolase class 2 resulting in a D-glyceraldehyde 3-phosphate. This compound can then either react in a reversible triosephosphate isomerase resulting in a dihydroxyacetone phosphate or react with a phosphate through a NAD dependent Glyceraldehyde 3-phosphate dehydrogenase resulting in a glyceric acid 1,3-biphosphate. This compound is desphosphorylated by a phosphoglycerate kinase resulting in a 3-phosphoglyceric acid.This compound in turn can either react with a 2,3-bisphosphoglycerate-independent phosphoglycerate mutase or a 2,3-bisphosphoglycerate-independent phosphoglycerate mutase resulting in a 2-phospho-D-glyceric acid. This compound interacts with an enolase resulting in a phosphoenolpyruvic acid and water. Phosphoenolpyruvic acid can react either through a AMP driven phosphoenoylpyruvate synthase or a ADP driven pyruvate kinase protein complex resulting in a pyruvic acid.
Pyruvic acid reacts with CoA through a NAD driven pyruvate dehydrogenase complex resulting in a carbon dioxide and a Acetyl-CoA which gets incorporated into the TCA cycle pathway.
|
Metabolite
Metabolic
|
|
|
SMP0438443![]() |
Sulfur Metabolism (Butanesulfonate)Bacteroides thetaiotaomicron VPI-5482
The sulfur metabolism pathway starts in three possible ways. The first is the uptake of sulfate through an active transport reaction via a sulfate transport system containing an ATP-binding protein which hydrolyses ATP. Sulfate is converted by the sulfate adenylyltransferase enzymatic complex to adenosine phosphosulfate through the addition of adenine from a molecule of ATP, along with one phosphate group. Adenosine phosphosulfate is further converted to phoaphoadenosine phosphosulfate through an ATP hydrolysis and dehydrogenation reaction by the adenylyl-sulfate kinase. Phoaphoadenosine phosphosulfate is finally dehydrogenated and converted to sulfite by phosphoadenosine phosphosulfate reductase. This reaction requires magnesium, and adenosine 3',5'-diphosphate is the bi-product. A thioredoxin is also oxidized. Sulfite can also be produced from the dehydrogenation of cyanide along with the conversion of thiosulfate to thiocyanate by the thiosulfate sulfurtransferase enzymatic complex. Sulfite next undergoes a series of reactions that lead to the production of pyruvic acid, which is a precursor for pathways such as gluconeogenesis. The first reaction in this series is the conversion of sulfite to hydrogen sulfide through hygrogenation and the deoxygenation of sulfite to form a water molecule. The reaction is catalyzed by the sulfite reductase [NADPH] flavoprotein alpha and beta components. Siroheme, 4Fe-4S, flavin mononucleotide, and FAD function as cofactors or prosthetic groups. Hydrogen sulfide next undergoes dehydrogenation in a reversible reaction to form L-Cysteine and acetic acid, via the cysteine synthase complex and the coenzyme pyridoxal 5'-phosphate. L-Cysteine is dehydrogenated and converted to 2-aminoacrylic acid (a bronsted acid) and hydrogen sulfide(which may be reused) by a larger enzymatic complex composed of cysteine synthase A/B, protein malY, cystathionine-β-lyase, and tryptophanase, along with the coenzyme pyridoxal 5'-phosphate. 2-aminoacrylic acid isomerizes to 2-iminopropanoate, which along with a water molecule and a hydrogen ion is lastly converted to pyruvic acid and ammonium in a spontaneous fashion. The second possible initial starting point for sulfur metabolism is the import of taurine(an alternate sulfur source) into the cytoplasm via the taurine ABC transporter complex. Taurine, oxoglutaric acid, and oxygen are converted to sulfite by the alpha-ketoglutarate-dependent taurine dioxygenase. Carbon dioxide, succinic acid, and aminoacetaldehyde are bi-products of this reaction. Sulfite next enters pyruvic acid synthesis as already described. The third variant of sulfur metabolism starts with the import of an alkyl sulfate, in this case 1-butanesulfonate, into the cytoplasm via an aliphatic sulfonate ABC transporter complex which hydrolyses ATP. 1-butanesulfonate is dehydrogenated and along with oxygen is converted to sulfite and betaine aldehyde by the FMNH2-dependent alkanesulfonate monooxygenase enzyme. Water and flavin mononucleotide(which is used in a subsequent reaction as a prosthetic group) are also produced. Sulfite is next converted to pyruvic acid by the process already described.
|
Metabolite
Metabolic
|
|
|
SMP0335795 |
Cardiolipin Biosynthesis CL(20:2(11Z,14Z)/20:4(5Z,8Z,11Z,14Z)/20:2(11Z,14Z)/18:4(6Z,9Z,12Z,15Z))Mus musculus
Cardiolipin (CL) is an important component of the inner mitochondrial membrane where it constitutes about 20% of the total lipid composition. It is essential for the optimal function of numerous enzymes that are involved in mitochondrial energy metabolism (Wikipedia). Cardiolipin biosynthesis occurs mainly in the mitochondria, but there also exists an alternative synthesis route for CDP-diacylglycerol that takes place in the endoplasmic reticulum. This second route may supplement this pathway. All membrane-localized enzymes are coloured dark green in the image. First, dihydroxyacetone phosphate (or glycerone phosphate) from glycolysis is used by the cytosolic enzyme glycerol-3-phosphate dehydrogenase [NAD(+)] to synthesize sn-glycerol 3-phosphate. Second, the mitochondrial outer membrane enzyme glycerol-3-phosphate acyltransferase esterifies an acyl-group to the sn-1 position of sn-glycerol 3-phosphate to form 1-acyl-sn-glycerol 3-phosphate (lysophosphatidic acid or LPA). Third, the enzyme 1-acyl-sn-glycerol-3-phosphate acyltransferase converts LPA into phosphatidic acid (PA or 1,2-diacyl-sn-glycerol 3-phosphate) by esterifying an acyl-group to the sn-2 position of the glycerol backbone. PA is then transferred to the inner mitochondrial membrane to continue cardiolipin synthesis. Fourth, magnesium-dependent phosphatidate cytidylyltransferase catalyzes the conversion of PA into CDP-diacylglycerol. Fifth, CDP-diacylglycerol--glycerol-3-phosphate 3-phosphatidyltransferase synthesizes phosphatidylglycerophosphate (PGP). Sixth, phosphatidylglycerophosphatase and protein-tyrosine phosphatase dephosphorylates PGP to form phosphatidylglycerol (PG). Last, cardiolipin synthase catalyzes the synthesis of cardiolipin by transferring a phosphatidyl group from a second CDP-diacylglycerol to PG. It requires a divalent metal cation cofactor.
|
Metabolite
Metabolic
|
|
|
SMP0335788 |
Cardiolipin Biosynthesis CL(20:2(11Z,14Z)/20:4(5Z,8Z,11Z,14Z)/18:4(6Z,9Z,12Z,15Z)/22:6(4Z,7Z,10Z,13Z,16Z,19Z))Mus musculus
Cardiolipin (CL) is an important component of the inner mitochondrial membrane where it constitutes about 20% of the total lipid composition. It is essential for the optimal function of numerous enzymes that are involved in mitochondrial energy metabolism (Wikipedia). Cardiolipin biosynthesis occurs mainly in the mitochondria, but there also exists an alternative synthesis route for CDP-diacylglycerol that takes place in the endoplasmic reticulum. This second route may supplement this pathway. All membrane-localized enzymes are coloured dark green in the image. First, dihydroxyacetone phosphate (or glycerone phosphate) from glycolysis is used by the cytosolic enzyme glycerol-3-phosphate dehydrogenase [NAD(+)] to synthesize sn-glycerol 3-phosphate. Second, the mitochondrial outer membrane enzyme glycerol-3-phosphate acyltransferase esterifies an acyl-group to the sn-1 position of sn-glycerol 3-phosphate to form 1-acyl-sn-glycerol 3-phosphate (lysophosphatidic acid or LPA). Third, the enzyme 1-acyl-sn-glycerol-3-phosphate acyltransferase converts LPA into phosphatidic acid (PA or 1,2-diacyl-sn-glycerol 3-phosphate) by esterifying an acyl-group to the sn-2 position of the glycerol backbone. PA is then transferred to the inner mitochondrial membrane to continue cardiolipin synthesis. Fourth, magnesium-dependent phosphatidate cytidylyltransferase catalyzes the conversion of PA into CDP-diacylglycerol. Fifth, CDP-diacylglycerol--glycerol-3-phosphate 3-phosphatidyltransferase synthesizes phosphatidylglycerophosphate (PGP). Sixth, phosphatidylglycerophosphatase and protein-tyrosine phosphatase dephosphorylates PGP to form phosphatidylglycerol (PG). Last, cardiolipin synthase catalyzes the synthesis of cardiolipin by transferring a phosphatidyl group from a second CDP-diacylglycerol to PG. It requires a divalent metal cation cofactor.
|
Metabolite
Metabolic
|
|
|
SMP0335793 |
Cardiolipin Biosynthesis CL(20:2(11Z,14Z)/20:4(5Z,8Z,11Z,14Z)/20:2(11Z,14Z)/18:1(9Z))Mus musculus
Cardiolipin (CL) is an important component of the inner mitochondrial membrane where it constitutes about 20% of the total lipid composition. It is essential for the optimal function of numerous enzymes that are involved in mitochondrial energy metabolism (Wikipedia). Cardiolipin biosynthesis occurs mainly in the mitochondria, but there also exists an alternative synthesis route for CDP-diacylglycerol that takes place in the endoplasmic reticulum. This second route may supplement this pathway. All membrane-localized enzymes are coloured dark green in the image. First, dihydroxyacetone phosphate (or glycerone phosphate) from glycolysis is used by the cytosolic enzyme glycerol-3-phosphate dehydrogenase [NAD(+)] to synthesize sn-glycerol 3-phosphate. Second, the mitochondrial outer membrane enzyme glycerol-3-phosphate acyltransferase esterifies an acyl-group to the sn-1 position of sn-glycerol 3-phosphate to form 1-acyl-sn-glycerol 3-phosphate (lysophosphatidic acid or LPA). Third, the enzyme 1-acyl-sn-glycerol-3-phosphate acyltransferase converts LPA into phosphatidic acid (PA or 1,2-diacyl-sn-glycerol 3-phosphate) by esterifying an acyl-group to the sn-2 position of the glycerol backbone. PA is then transferred to the inner mitochondrial membrane to continue cardiolipin synthesis. Fourth, magnesium-dependent phosphatidate cytidylyltransferase catalyzes the conversion of PA into CDP-diacylglycerol. Fifth, CDP-diacylglycerol--glycerol-3-phosphate 3-phosphatidyltransferase synthesizes phosphatidylglycerophosphate (PGP). Sixth, phosphatidylglycerophosphatase and protein-tyrosine phosphatase dephosphorylates PGP to form phosphatidylglycerol (PG). Last, cardiolipin synthase catalyzes the synthesis of cardiolipin by transferring a phosphatidyl group from a second CDP-diacylglycerol to PG. It requires a divalent metal cation cofactor.
|
Metabolite
Metabolic
|
|
|
SMP0419468![]() |
Oxidative PhosphorylationBacteroides stercoris ATCC 43183
The process of oxidative phosphorylation involves multiple interactions of ubiquinone with succinic acid, resulting in a fumaric acid and ubiquinol.
Ubiquinone interacts with succinic acid through a succinate:quinone oxidoreductase resulting in a fumaric acid an ubiquinol. This enzyme has various cofactors, ferroheme b, 2FE-2S, FAD, and 3Fe-4S iron-sulfur cluster.
Then 2 ubiquinol interact with oxygen and 4 hydrogen ion through a cytochrome bd-I terminal oxidase resulting in a 4 hydrogen ion transferred into the periplasmic space, 2 water returned into the cytoplasm and 2 ubiquinone, which stay in the inner membrane.
The ubiquinone interacts with succinic acid through a succinate:quinone oxidoreductase resulting in a fumaric acid an ubiquinol.
Then 2 ubiquinol interacts with oxygen and 4 hydrogen ion through a cytochrome bd-II terminal oxidase resulting in a 4 hydrogen ion transferred into the periplasmic space, 2 water returned into the cytoplasm and 2 ubiquinone, which stay in the inner membrane.
The ubiquinone interacts with succinic acid through a succinate:quinone oxidoreductase resulting in a fumaric acid an ubiquinol.
The 2 ubiquinol interact with oxygen and 8 hydrogen ion through a cytochrome bo terminal oxidase resulting in a 8 hydrogen ion transferred into the periplasmic space, 2 water returned into the cytoplasm and 2 ubiquinone, which stays in the inner membrane.
The ubiquinone then interacts with 5 hydrogen ion through a NADH dependent ubiquinone oxidoreductase I resulting in NAD, hydrogen ion released into the periplasmic space and an ubiquinol.
The ubiquinol is then processed reacting with oxygen, and 4 hydrogen through a ion cytochrome bd-I terminal oxidase resulting in 4 hydrogen ions released into the periplasmic space, 2 water molecules into the cytoplasm and 2 ubiquinones.
The ubiquinone then interacts with 5 hydrogen ion through a NADH dependent ubiquinone oxidoreductase I resulting in NAD, hydrogen ion released into the periplasmic space and an ubiquinol.
The 2 ubiquinol interact with oxygen and 8 hydrogen ion through a cytochrome bo terminal oxidase resulting in a 8 hydrogen ion transferred into the periplasmic space, 2 water returned into the cytoplasm and 2 ubiquinone, which stays in the inner membrane.
|
Metabolite
Metabolic
|
|
|
SMP0440227![]() |
beta-Alanine MetabolismNeisseria meningitidis Z2491
Beta-Alanine metabolism starts as a product of aspartate metabolism. Aspartate is decarboxylated by aspartate 1-decarboxylase, releasing carbon dioxide and beta-alanine. Beta-Alanine is then metabolized through a pantothenate synthease resulting in pantothenic acid. Pantothenic acid then undergoes phosphorylation through an ATP-driven pantothenate kinase, resulting in D-4-phosphopantothenate. Pantothenate, vitamin B5, is a precursor for synthesis of 4'-phosphopantetheine moiety of coenzyme A and acyl carrier protein. Plants and microorganisms can synthesize pantothenate de novo, but animals must obtain it from diet. Enzymes of beta-alanine metabolism are targets for anti-microbial drugs.
|
Metabolite
Metabolic
|
||
SMP0440231![]() |
Cardiolipin Biosynthesis CL(a-13:0/i-16:0/i-22:0/i-17:0)[rac]Homo sapiens
Cardiolipin (CL) is an important component of the inner mitochondrial membrane where it constitutes about 20% of the total lipid composition. It is essential for the optimal function of numerous enzymes that are involved in mitochondrial energy metabolism (Wikipedia). Cardiolipin biosynthesis occurs mainly in the mitochondria, but there also exists an alternative synthesis route for CDP-diacylglycerol that takes place in the endoplasmic reticulum. This second route may supplement this pathway. All membrane-localized enzymes are coloured dark green in the image. First, dihydroxyacetone phosphate (or glycerone phosphate) from glycolysis is used by the cytosolic enzyme glycerol-3-phosphate dehydrogenase [NAD(+)] to synthesize sn-glycerol 3-phosphate. Second, the mitochondrial outer membrane enzyme glycerol-3-phosphate acyltransferase esterifies an acyl-group to the sn-1 position of sn-glycerol 3-phosphate to form 1-acyl-sn-glycerol 3-phosphate (lysophosphatidic acid or LPA). Third, the enzyme 1-acyl-sn-glycerol-3-phosphate acyltransferase converts LPA into phosphatidic acid (PA or 1,2-diacyl-sn-glycerol 3-phosphate) by esterifying an acyl-group to the sn-2 position of the glycerol backbone. PA is then transferred to the inner mitochondrial membrane to continue cardiolipin synthesis. Fourth, magnesium-dependent phosphatidate cytidylyltransferase catalyzes the conversion of PA into CDP-diacylglycerol. Fifth, CDP-diacylglycerol--glycerol-3-phosphate 3-phosphatidyltransferase synthesizes phosphatidylglycerophosphate (PGP). Sixth, phosphatidylglycerophosphatase and protein-tyrosine phosphatase dephosphorylates PGP to form phosphatidylglycerol (PG). Last, cardiolipin synthase catalyzes the synthesis of cardiolipin by transferring a phosphatidyl group from a second CDP-diacylglycerol to PG. It requires a divalent metal cation cofactor.
|
Metabolite
Metabolic
|
|
|
SMP0438501![]() |
Fructose MetabolismCampylobacter fetus subsp. fetus 82-40
Fructose metabolism begins with the transport of Beta-D-fructofuranose through a fructose PTS permease, resulting in a Beta-D-fructofuranose 1-phosphate. This compound is phosphorylated by an ATP driven 1-phosphofructokinase resulting in a fructose 1,6-biphosphate. This compound can either react with a fructose bisphosphate aldolase class 1 resulting in D-glyceraldehyde 3-phosphate and a dihydroxyacetone phosphate or through a fructose biphosphate aldolase class 2 resulting in a D-glyceraldehyde 3-phosphate. This compound can then either react in a reversible triosephosphate isomerase resulting in a dihydroxyacetone phosphate or react with a phosphate through a NAD dependent Glyceraldehyde 3-phosphate dehydrogenase resulting in a glyceric acid 1,3-biphosphate. This compound is desphosphorylated by a phosphoglycerate kinase resulting in a 3-phosphoglyceric acid.This compound in turn can either react with a 2,3-bisphosphoglycerate-independent phosphoglycerate mutase or a 2,3-bisphosphoglycerate-independent phosphoglycerate mutase resulting in a 2-phospho-D-glyceric acid. This compound interacts with an enolase resulting in a phosphoenolpyruvic acid and water. Phosphoenolpyruvic acid can react either through a AMP driven phosphoenoylpyruvate synthase or a ADP driven pyruvate kinase protein complex resulting in a pyruvic acid.
Pyruvic acid reacts with CoA through a NAD driven pyruvate dehydrogenase complex resulting in a carbon dioxide and a Acetyl-CoA which gets incorporated into the TCA cycle pathway.
|
Metabolite
Metabolic
|
|
|
SMP0438480![]() |
Oxidative PhosphorylationBradyrhizobium elkanii USDA 76
The process of oxidative phosphorylation involves multiple interactions of ubiquinone with succinic acid, resulting in a fumaric acid and ubiquinol.
Ubiquinone interacts with succinic acid through a succinate:quinone oxidoreductase resulting in a fumaric acid an ubiquinol. This enzyme has various cofactors, ferroheme b, 2FE-2S, FAD, and 3Fe-4S iron-sulfur cluster.
Then 2 ubiquinol interact with oxygen and 4 hydrogen ion through a cytochrome bd-I terminal oxidase resulting in a 4 hydrogen ion transferred into the periplasmic space, 2 water returned into the cytoplasm and 2 ubiquinone, which stay in the inner membrane.
The ubiquinone interacts with succinic acid through a succinate:quinone oxidoreductase resulting in a fumaric acid an ubiquinol.
Then 2 ubiquinol interacts with oxygen and 4 hydrogen ion through a cytochrome bd-II terminal oxidase resulting in a 4 hydrogen ion transferred into the periplasmic space, 2 water returned into the cytoplasm and 2 ubiquinone, which stay in the inner membrane.
The ubiquinone interacts with succinic acid through a succinate:quinone oxidoreductase resulting in a fumaric acid an ubiquinol.
The 2 ubiquinol interact with oxygen and 8 hydrogen ion through a cytochrome bo terminal oxidase resulting in a 8 hydrogen ion transferred into the periplasmic space, 2 water returned into the cytoplasm and 2 ubiquinone, which stays in the inner membrane.
The ubiquinone then interacts with 5 hydrogen ion through a NADH dependent ubiquinone oxidoreductase I resulting in NAD, hydrogen ion released into the periplasmic space and an ubiquinol.
The ubiquinol is then processed reacting with oxygen, and 4 hydrogen through a ion cytochrome bd-I terminal oxidase resulting in 4 hydrogen ions released into the periplasmic space, 2 water molecules into the cytoplasm and 2 ubiquinones.
The ubiquinone then interacts with 5 hydrogen ion through a NADH dependent ubiquinone oxidoreductase I resulting in NAD, hydrogen ion released into the periplasmic space and an ubiquinol.
The 2 ubiquinol interact with oxygen and 8 hydrogen ion through a cytochrome bo terminal oxidase resulting in a 8 hydrogen ion transferred into the periplasmic space, 2 water returned into the cytoplasm and 2 ubiquinone, which stays in the inner membrane.
|
Metabolite
Metabolic
|
Showing 366911 -
366920 of 367451 pathways