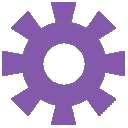
Browsing Pathways
Showing 369901 -
369910 of 605359 pathways
PathBank ID | Pathway Name and Description | Pathway Class | Chemical Compounds | Proteins |
---|---|---|---|---|
SMP0443537![]() |
Glycerol MetabolismFusobacterium periodonticum 1_1_41FAA
Glycerol metabolism starts with glycerol is introduced into the cytoplasm through a glycerol channel GlpF Glycerol is then phosphorylated through an ATP mediated glycerol kinase resulting in a Glycerol 3-phosphate. This compound can also be obtained through a glycerophosphodiester reacting with water through a glycerophosphoryl diester phosphodiesterase or it can also be introduced into the cytoplasm through a glycerol-3-phosphate:phosphate antiporter.
Glycerol 3-phosphate is then metabolized into a dihydroxyacetone phosphate in both aerobic or anaerobic conditions. In anaerobic conditions the metabolism is done through the reaction of glycerol 3-phosphate with a menaquinone mediated by a glycerol-3-phosphate dehydrogenase protein complex. In aerobic conditions, the metabolism is done through the reaction of glycerol 3-phosphate with ubiquinone mediated by a glycerol-3-phosphate dehydrogenase [NAD(P]+].
Dihydroxyacetone phosphate is then introduced into the fructose metabolism by turning a dihydroxyacetone into an isomer through a triosephosphate isomerase resulting in a D-glyceraldehyde 3-phosphate which in turn reacts with a phosphate through a NAD dependent Glyceraldehyde 3-phosphate dehydrogenase resulting in a glyceric acid 1,3-biphosphate. This compound is desphosphorylated by a phosphoglycerate kinase resulting in a 3-phosphoglyceric acid.This compound in turn can either react with a 2,3-bisphosphoglycerate-independent phosphoglycerate mutase or a 2,3-bisphosphoglycerate-independent phosphoglycerate mutase resulting in a 2-phospho-D-glyceric acid. This compound interacts with an enolase resulting in a phosphoenolpyruvic acid and water. Phosphoenolpyruvic acid can react either through a AMP driven phosphoenoylpyruvate synthase or a ADP driven pyruvate kinase protein complex resulting in a pyruvic acid. Pyruvic acid reacts with CoA through a NAD driven pyruvate dehydrogenase complex resulting in a carbon dioxide and a Acetyl-CoA which gets incorporated into the TCA cycle pathway.
|
Metabolite
Metabolic
|
|
|
SMP0443534![]() |
Propanoate MetabolismAcidaminococcus intestini RyC-MR95
Starting from L-threonine, this compound is deaminated through a threonine deaminase resulting in a hydrogen ion, a water molecule and a (2z)-2-aminobut-2-enoate. The latter compound then isomerizes to a 2-iminobutanoate, This compound then reacts spontaneously with hydrogen ion and a water molecule resulting in a ammonium and a 2-Ketobutyric acid. The latter compound interacts with CoA through a pyruvate formate-lyase / 2-ketobutyrate formate-lyase resulting in a formic acid and a propionyl-CoA.
Propionyl-CoA can then be processed either into a 2-methylcitric acid or into a propanoyl phosphate. Propionyl-CoA interacts with oxalacetic acid and a water molecule through a 2-methylcitrate synthase resulting in a hydrogen ion, a CoA and a 2-Methylcitric acid.The latter compound is dehydrated through a 2-methylcitrate dehydratase resulting in a water molecule and cis-2-methylaconitate. The latter compound is then dehydrated by a bifunctional aconitate hydratase 2 and 2-methylisocitrate dehydratase resulting in a water molecule and methylisocitric acid. The latter compound is then processed by 2-methylisocitrate lyase resulting in a release of succinic acid and pyruvic acid. Succinic acid can then interact with a propionyl-CoA through a propionyl-CoA:succinate CoA transferase resulting in a propionic acid and a succinyl CoA. Succinyl-CoA is then isomerized through a methylmalonyl-CoA mutase resulting in a methylmalonyl-CoA. This compound is then decarboxylated through a methylmalonyl-CoA decarboxylase resulting in a release of Carbon dioxide and Propionyl-CoA. Propionyl-CoA interacts with a phosphate through a phosphate acetyltransferase / phosphate propionyltransferase resulting in a CoA and a propanoyl phosphate. Propionyl-CoA can react with a phosphate through a phosphate acetyltransferase / phosphate propionyltransferase resulting in a CoA and a propanoyl phosphate. The latter compound is then dephosphorylated through a ADP driven acetate kinase/propionate kinase protein complex resulting in an ATP and Propionic acid. Propionic acid can be processed by a reaction with CoA through a ATP-driven propionyl-CoA synthetase resulting in a pyrophosphate, an AMP and a propionyl-CoA.
|
Metabolite
Metabolic
|
|
|
SMP0423528![]() |
Phenylalanine MetabolismFusobacterium periodonticum 1_1_41FAA
The pathways of the metabolism of phenylalaline begins with the conversion of chorismate to prephenate through a P-protein (chorismate mutase:pheA). Prephenate then interacts with a hydrogen ion through the same previous enzyme resulting in a release of carbon dioxide, water and a phenolpyruvic acid. Three enzymes those enconde by tyrB, aspC and ilvE are involved in catalyzing the third step of these pathways, all three can contribute to the synthesis of phenylalanine: only tyrB and aspC contribute to biosynthesis of tyrosine.
Phenolpyruvic acid can also be obtained from a reversivle reaction with ammonia, a reduced acceptor and a D-amino acid dehydrogenase, resulting in a water, an acceptor and a D-phenylalanine, which can be then transported into the periplasmic space by aromatic amino acid exporter.
L-phenylalanine also interacts in two reversible reactions, one involved with oxygen through a catalase peroxidase resulting in a carbon dioxide and 2-phenylacetamide. The other reaction involved an interaction with oxygen through a phenylalanine aminotransferase resulting in a oxoglutaric acid and phenylpyruvic acid.
L-phenylalanine can be imported into the cytoplasm through an aromatic amino acid:H+ symporter AroP.
The compound can also be imported into the periplasmic space through a transporter: L-amino acid efflux transporter.
|
Metabolite
Metabolic
|
||
SMP0338613 |
Cardiolipin Biosynthesis CL(22:6(4Z,7Z,10Z,13Z,16Z,19Z)/20:5(5Z,8Z,11Z,14Z,17Z)/18:1(9Z)/20:5(5Z,8Z,11Z,14Z,17Z))Mus musculus
Cardiolipin (CL) is an important component of the inner mitochondrial membrane where it constitutes about 20% of the total lipid composition. It is essential for the optimal function of numerous enzymes that are involved in mitochondrial energy metabolism (Wikipedia). Cardiolipin biosynthesis occurs mainly in the mitochondria, but there also exists an alternative synthesis route for CDP-diacylglycerol that takes place in the endoplasmic reticulum. This second route may supplement this pathway. All membrane-localized enzymes are coloured dark green in the image. First, dihydroxyacetone phosphate (or glycerone phosphate) from glycolysis is used by the cytosolic enzyme glycerol-3-phosphate dehydrogenase [NAD(+)] to synthesize sn-glycerol 3-phosphate. Second, the mitochondrial outer membrane enzyme glycerol-3-phosphate acyltransferase esterifies an acyl-group to the sn-1 position of sn-glycerol 3-phosphate to form 1-acyl-sn-glycerol 3-phosphate (lysophosphatidic acid or LPA). Third, the enzyme 1-acyl-sn-glycerol-3-phosphate acyltransferase converts LPA into phosphatidic acid (PA or 1,2-diacyl-sn-glycerol 3-phosphate) by esterifying an acyl-group to the sn-2 position of the glycerol backbone. PA is then transferred to the inner mitochondrial membrane to continue cardiolipin synthesis. Fourth, magnesium-dependent phosphatidate cytidylyltransferase catalyzes the conversion of PA into CDP-diacylglycerol. Fifth, CDP-diacylglycerol--glycerol-3-phosphate 3-phosphatidyltransferase synthesizes phosphatidylglycerophosphate (PGP). Sixth, phosphatidylglycerophosphatase and protein-tyrosine phosphatase dephosphorylates PGP to form phosphatidylglycerol (PG). Last, cardiolipin synthase catalyzes the synthesis of cardiolipin by transferring a phosphatidyl group from a second CDP-diacylglycerol to PG. It requires a divalent metal cation cofactor.
|
Metabolite
Metabolic
|
|
|
SMP0338618 |
Cardiolipin Biosynthesis CL(22:6(4Z,7Z,10Z,13Z,16Z,19Z)/20:5(5Z,8Z,11Z,14Z,17Z)/18:2(9Z,12Z)/16:0)Mus musculus
Cardiolipin (CL) is an important component of the inner mitochondrial membrane where it constitutes about 20% of the total lipid composition. It is essential for the optimal function of numerous enzymes that are involved in mitochondrial energy metabolism (Wikipedia). Cardiolipin biosynthesis occurs mainly in the mitochondria, but there also exists an alternative synthesis route for CDP-diacylglycerol that takes place in the endoplasmic reticulum. This second route may supplement this pathway. All membrane-localized enzymes are coloured dark green in the image. First, dihydroxyacetone phosphate (or glycerone phosphate) from glycolysis is used by the cytosolic enzyme glycerol-3-phosphate dehydrogenase [NAD(+)] to synthesize sn-glycerol 3-phosphate. Second, the mitochondrial outer membrane enzyme glycerol-3-phosphate acyltransferase esterifies an acyl-group to the sn-1 position of sn-glycerol 3-phosphate to form 1-acyl-sn-glycerol 3-phosphate (lysophosphatidic acid or LPA). Third, the enzyme 1-acyl-sn-glycerol-3-phosphate acyltransferase converts LPA into phosphatidic acid (PA or 1,2-diacyl-sn-glycerol 3-phosphate) by esterifying an acyl-group to the sn-2 position of the glycerol backbone. PA is then transferred to the inner mitochondrial membrane to continue cardiolipin synthesis. Fourth, magnesium-dependent phosphatidate cytidylyltransferase catalyzes the conversion of PA into CDP-diacylglycerol. Fifth, CDP-diacylglycerol--glycerol-3-phosphate 3-phosphatidyltransferase synthesizes phosphatidylglycerophosphate (PGP). Sixth, phosphatidylglycerophosphatase and protein-tyrosine phosphatase dephosphorylates PGP to form phosphatidylglycerol (PG). Last, cardiolipin synthase catalyzes the synthesis of cardiolipin by transferring a phosphatidyl group from a second CDP-diacylglycerol to PG. It requires a divalent metal cation cofactor.
|
Metabolite
Metabolic
|
|
|
SMP0338611 |
Cardiolipin Biosynthesis CL(22:6(4Z,7Z,10Z,13Z,16Z,19Z)/20:5(5Z,8Z,11Z,14Z,17Z)/18:1(9Z)/20:3(11Z,14Z,17Z))Mus musculus
Cardiolipin (CL) is an important component of the inner mitochondrial membrane where it constitutes about 20% of the total lipid composition. It is essential for the optimal function of numerous enzymes that are involved in mitochondrial energy metabolism (Wikipedia). Cardiolipin biosynthesis occurs mainly in the mitochondria, but there also exists an alternative synthesis route for CDP-diacylglycerol that takes place in the endoplasmic reticulum. This second route may supplement this pathway. All membrane-localized enzymes are coloured dark green in the image. First, dihydroxyacetone phosphate (or glycerone phosphate) from glycolysis is used by the cytosolic enzyme glycerol-3-phosphate dehydrogenase [NAD(+)] to synthesize sn-glycerol 3-phosphate. Second, the mitochondrial outer membrane enzyme glycerol-3-phosphate acyltransferase esterifies an acyl-group to the sn-1 position of sn-glycerol 3-phosphate to form 1-acyl-sn-glycerol 3-phosphate (lysophosphatidic acid or LPA). Third, the enzyme 1-acyl-sn-glycerol-3-phosphate acyltransferase converts LPA into phosphatidic acid (PA or 1,2-diacyl-sn-glycerol 3-phosphate) by esterifying an acyl-group to the sn-2 position of the glycerol backbone. PA is then transferred to the inner mitochondrial membrane to continue cardiolipin synthesis. Fourth, magnesium-dependent phosphatidate cytidylyltransferase catalyzes the conversion of PA into CDP-diacylglycerol. Fifth, CDP-diacylglycerol--glycerol-3-phosphate 3-phosphatidyltransferase synthesizes phosphatidylglycerophosphate (PGP). Sixth, phosphatidylglycerophosphatase and protein-tyrosine phosphatase dephosphorylates PGP to form phosphatidylglycerol (PG). Last, cardiolipin synthase catalyzes the synthesis of cardiolipin by transferring a phosphatidyl group from a second CDP-diacylglycerol to PG. It requires a divalent metal cation cofactor.
|
Metabolite
Metabolic
|
|
|
SMP0443547![]() |
Cardiolipin Biosynthesis CL(i-13:0/i-16:0/a-13:0/a-25:0)[rac]Homo sapiens
Cardiolipin (CL) is an important component of the inner mitochondrial membrane where it constitutes about 20% of the total lipid composition. It is essential for the optimal function of numerous enzymes that are involved in mitochondrial energy metabolism (Wikipedia). Cardiolipin biosynthesis occurs mainly in the mitochondria, but there also exists an alternative synthesis route for CDP-diacylglycerol that takes place in the endoplasmic reticulum. This second route may supplement this pathway. All membrane-localized enzymes are coloured dark green in the image. First, dihydroxyacetone phosphate (or glycerone phosphate) from glycolysis is used by the cytosolic enzyme glycerol-3-phosphate dehydrogenase [NAD(+)] to synthesize sn-glycerol 3-phosphate. Second, the mitochondrial outer membrane enzyme glycerol-3-phosphate acyltransferase esterifies an acyl-group to the sn-1 position of sn-glycerol 3-phosphate to form 1-acyl-sn-glycerol 3-phosphate (lysophosphatidic acid or LPA). Third, the enzyme 1-acyl-sn-glycerol-3-phosphate acyltransferase converts LPA into phosphatidic acid (PA or 1,2-diacyl-sn-glycerol 3-phosphate) by esterifying an acyl-group to the sn-2 position of the glycerol backbone. PA is then transferred to the inner mitochondrial membrane to continue cardiolipin synthesis. Fourth, magnesium-dependent phosphatidate cytidylyltransferase catalyzes the conversion of PA into CDP-diacylglycerol. Fifth, CDP-diacylglycerol--glycerol-3-phosphate 3-phosphatidyltransferase synthesizes phosphatidylglycerophosphate (PGP). Sixth, phosphatidylglycerophosphatase and protein-tyrosine phosphatase dephosphorylates PGP to form phosphatidylglycerol (PG). Last, cardiolipin synthase catalyzes the synthesis of cardiolipin by transferring a phosphatidyl group from a second CDP-diacylglycerol to PG. It requires a divalent metal cation cofactor.
|
Metabolite
Metabolic
|
|
|
SMP0423110![]() |
Purine Nucleotides De Novo BiosynthesisAlistipes putredinis DSM 17216
The biosynthesis of purine nucleotides is a complex process that begins with a phosphoribosyl pyrophosphate. This compound interacts with water and L-glutamine through a
amidophosphoribosyl transferase resulting in a pyrophosphate, L-glutamic acid and a 5-phosphoribosylamine. The latter compound proceeds to interact with a glycine through an ATP driven phosphoribosylamine-glycine ligase resulting in the addition of glycine to the compound. This reaction releases an ADP, a phosphate, a hydrogen ion and a N1-(5-phospho-β-D-ribosyl)glycinamide. The latter compound interacts with formic acid, through an ATP driven phosphoribosylglycinamide formyltransferase 2 resulting in a phosphate, an ADP, a hydrogen ion and a 5-phosphoribosyl-N-formylglycinamide. The latter compound interacts with L-glutamine, and water through an ATP-driven
phosphoribosylformylglycinamide synthetase resulting in a release of a phosphate, an ADP, a hydrogen ion, a L-glutamic acid and a 2-(formamido)-N1-(5-phospho-D-ribosyl)acetamidine. The latter compound interacts with an ATP driven phosphoribosylformylglycinamide cyclo-ligase resulting in a release of ADP, a phosphate, a hydrogen ion and a 5-aminoimidazole ribonucleotide. The latter compound interacts with a hydrogen carbonate through an ATP driven N5-carboxyaminoimidazole ribonucleotide synthetase resulting in a release of a phosphate, an ADP, a hydrogen ion and a N5-carboxyaminoimidazole ribonucleotide.The latter compound then interacts with a N5-carboxyaminoimidazole ribonucleotide mutase resulting in a 5-amino-1-(5-phospho-D-ribosyl)imidazole-4-carboxylate. This compound interacts with an L-aspartic acid through an ATP driven phosphoribosylaminoimidazole-succinocarboxamide synthase resulting in a phosphate, an ADP, a hydrogen ion and a SAICAR. SAICAR interacts with an adenylosuccinate lyase resulting in a fumaric acid and an AICAR. AICAR interacts with a formyltetrahydrofolate through a AICAR transformylase / IMP cyclohydrolase resulting in a release of a tetrahydropterol mono-l-glutamate and a FAICAR. The latter compound, FAICAR, interacts in a reversible reaction through a AICAR transformylase / IMP cyclohydrolase resulting in a release of water and Inosinic acid.
Inosinic acid can be metabolized to produce dGTP and dATP three different methods each.
dGTP:
Inosinic acid, water and NAD are processed by IMP dehydrogenase resulting in a release of NADH, a hydrogen ion and Xanthylic acid. Xanthylic acid interacts with L-glutamine, and water through an ATP driven GMP synthetase resulting in pyrophosphate, AMP, L-glutamic acid, a hydrogen ion and Guanosine monophosphate. The latter compound is the phosphorylated by reacting with an ATP driven guanylate kinase resulting in a release of ADP and a Gaunosine diphosphate. Guanosine diphosphate can be metabolized in three different ways:
1.-Guanosine diphosphate is phosphorylated by an ATP-driven nucleoside diphosphate kinase resulting in an ADP and a Guanosine triphosphate. This compound interacts with a reduced flavodoxin protein through a ribonucleoside-triphosphate reductase resulting in a oxidized flavodoxin a water moleculer and a dGTP
2.-Guanosine diphosphate interacts with a reduced NrdH glutaredoxin-like proteins through a ribonucleoside-diphosphate reductase 2 resulting in the release of an oxidized NrdH glutaredoxin-like protein, a water molecule and a dGDP. The dGDP is then phosphorylated by interacting with an ATP-driven nucleoside diphosphate kinase resulting in an ADP and dGTP.
3.-Guanosine diphosphate interacts with a reduced thioredoxin ribonucleoside diphosphate reductase 1 resulting in a release of a water molecule, an oxidized thioredoxin and a dGDP. The dGDP is then phosphorylated by interacting with an ATP-driven nucleoside diphosphate kinase resulting in an ADP and dGTP.
dATP:
Inosinic acid interacts with L-aspartic acid through an GTP driven adenylosuccinate synthase results in the release of GDP, a hydrogen ion, a phosphate and N(6)-(1,2-dicarboxyethyl)AMP. The latter compound is then cleaved by a adenylosuccinate lyase resulting in a fumaric acid and an Adenosine monophosphate. This compound is then phosphorylated by an adenylate kinase resulting in the release of ATP and an adenosine diphosphate. Adenosine diphosphate can be metabolized in three different ways:
1.-Adenosine diphosphate is involved in a reversible reaction by interacting with a hydrogen ion and a phosphate through a ATP synthase / thiamin triphosphate synthase resulting in a hydrogen ion, a water molecule and an Adenosine triphosphate. The adenosine triphosphate interacts with a reduced flavodoxin through a ribonucleoside-triphosphate reductase resulting in an oxidized flavodoxin, a water molecule and a dATP
2.- Adenosine diphosphate interacts with an reduced thioredoxin through a ribonucleoside diphosphate reductase 1 resulting in a release of a water molecule, a oxidized thioredoxin and a dADP. The dADP is then phosphorylated by a nucleoside diphosphate kinase resulting in the release of ADP and a dATP
3.- Adenosine diphosphate interacts with an reduced NrdH glutaredoxin-like protein through a ribonucleoside diphosphate reductase 2 resulting in a release of a water molecule, a oxidized glutaredoxin-like protein and a dADP. The dADP is then phosphorylated by a nucleoside diphosphate kinase resulting in the release of ADP and a dATP
|
Metabolite
Metabolic
|
|
|
SMP0423232![]() |
Sulfur Metabolism (Butanesulfonate)Parabacteroides sp. D13
The sulfur metabolism pathway starts in three possible ways. The first is the uptake of sulfate through an active transport reaction via a sulfate transport system containing an ATP-binding protein which hydrolyses ATP. Sulfate is converted by the sulfate adenylyltransferase enzymatic complex to adenosine phosphosulfate through the addition of adenine from a molecule of ATP, along with one phosphate group. Adenosine phosphosulfate is further converted to phoaphoadenosine phosphosulfate through an ATP hydrolysis and dehydrogenation reaction by the adenylyl-sulfate kinase. Phoaphoadenosine phosphosulfate is finally dehydrogenated and converted to sulfite by phosphoadenosine phosphosulfate reductase. This reaction requires magnesium, and adenosine 3',5'-diphosphate is the bi-product. A thioredoxin is also oxidized. Sulfite can also be produced from the dehydrogenation of cyanide along with the conversion of thiosulfate to thiocyanate by the thiosulfate sulfurtransferase enzymatic complex. Sulfite next undergoes a series of reactions that lead to the production of pyruvic acid, which is a precursor for pathways such as gluconeogenesis. The first reaction in this series is the conversion of sulfite to hydrogen sulfide through hygrogenation and the deoxygenation of sulfite to form a water molecule. The reaction is catalyzed by the sulfite reductase [NADPH] flavoprotein alpha and beta components. Siroheme, 4Fe-4S, flavin mononucleotide, and FAD function as cofactors or prosthetic groups. Hydrogen sulfide next undergoes dehydrogenation in a reversible reaction to form L-Cysteine and acetic acid, via the cysteine synthase complex and the coenzyme pyridoxal 5'-phosphate. L-Cysteine is dehydrogenated and converted to 2-aminoacrylic acid (a bronsted acid) and hydrogen sulfide(which may be reused) by a larger enzymatic complex composed of cysteine synthase A/B, protein malY, cystathionine-β-lyase, and tryptophanase, along with the coenzyme pyridoxal 5'-phosphate. 2-aminoacrylic acid isomerizes to 2-iminopropanoate, which along with a water molecule and a hydrogen ion is lastly converted to pyruvic acid and ammonium in a spontaneous fashion. The second possible initial starting point for sulfur metabolism is the import of taurine(an alternate sulfur source) into the cytoplasm via the taurine ABC transporter complex. Taurine, oxoglutaric acid, and oxygen are converted to sulfite by the alpha-ketoglutarate-dependent taurine dioxygenase. Carbon dioxide, succinic acid, and aminoacetaldehyde are bi-products of this reaction. Sulfite next enters pyruvic acid synthesis as already described. The third variant of sulfur metabolism starts with the import of an alkyl sulfate, in this case 1-butanesulfonate, into the cytoplasm via an aliphatic sulfonate ABC transporter complex which hydrolyses ATP. 1-butanesulfonate is dehydrogenated and along with oxygen is converted to sulfite and betaine aldehyde by the FMNH2-dependent alkanesulfonate monooxygenase enzyme. Water and flavin mononucleotide(which is used in a subsequent reaction as a prosthetic group) are also produced. Sulfite is next converted to pyruvic acid by the process already described.
|
Metabolite
Metabolic
|
|
|
SMP0443071![]() |
Sulfur Metabolism (Methanesulfonate)Parabacteroides sp. 2_1_7
The sulfur metabolism pathway starts in three possible ways. The first is the uptake of sulfate through an active transport reaction via a sulfate transport system containing an ATP-binding protein which hydrolyses ATP. Sulfate is converted by the sulfate adenylyltransferase enzymatic complex to adenosine phosphosulfate through the addition of adenine from a molecule of ATP, along with one phosphate group. Adenosine phosphosulfate is further converted to phoaphoadenosine phosphosulfate through an ATP hydrolysis and dehydrogenation reaction by the adenylyl-sulfate kinase. Phoaphoadenosine phosphosulfate is finally dehydrogenated and converted to sulfite by phosphoadenosine phosphosulfate reductase. This reaction requires magnesium, and adenosine 3',5'-diphosphate is the bi-product. A thioredoxin is also oxidized. Sulfite can also be produced from the dehydrogenation of cyanide along with the conversion of thiosulfate to thiocyanate by the thiosulfate sulfurtransferase enzymatic complex. Sulfite next undergoes a series of reactions that lead to the production of pyruvic acid, which is a precursor for pathways such as gluconeogenesis. The first reaction in this series is the conversion of sulfite to hydrogen sulfide through hygrogenation and the deoxygenation of sulfite to form a water molecule. The reaction is catalyzed by the sulfite reductase [NADPH] flavoprotein alpha and beta components. Siroheme, 4Fe-4S, flavin mononucleotide, and FAD function as cofactors or prosthetic groups. Hydrogen sulfide next undergoes dehydrogenation in a reversible reaction to form L-Cysteine and acetic acid, via the cysteine synthase complex and the coenzyme pyridoxal 5'-phosphate. L-Cysteine is dehydrogenated and converted to 2-aminoacrylic acid (a bronsted acid) and hydrogen sulfide(which may be reused) by a larger enzymatic complex composed of cysteine synthase A/B, protein malY, cystathionine-β-lyase, and tryptophanase, along with the coenzyme pyridoxal 5'-phosphate. 2-aminoacrylic acid isomerizes to 2-iminopropanoate, which along with a water molecule and a hydrogen ion is lastly converted to pyruvic acid and ammonium in a spontaneous fashion. The second possible initial starting point for sulfur metabolism is the import of taurine(an alternate sulfur source) into the cytoplasm via the taurine ABC transporter complex. Taurine, oxoglutaric acid, and oxygen are converted to sulfite by the alpha-ketoglutarate-dependent taurine dioxygenase. Carbon dioxide, succinic acid, and aminoacetaldehyde are bi-products of this reaction. Sulfite next enters pyruvic acid synthesis as already described. The third variant of sulfur metabolism starts with the import of an alkyl sulfate, in this case 1-butanesulfonate, into the cytoplasm via an aliphatic sulfonate ABC transporter complex which hydrolyses ATP. 1-butanesulfonate is dehydrogenated and along with oxygen is converted to sulfite and betaine aldehyde by the FMNH2-dependent alkanesulfonate monooxygenase enzyme. Water and flavin mononucleotide(which is used in a subsequent reaction as a prosthetic group) are also produced. Sulfite is next converted to pyruvic acid by the process already described.
|
Metabolite
Metabolic
|
|
Showing 369901 -
369910 of 369998 pathways